Abstract
Background:
Traumatic brain injury (TBI), even single-impact mild TBI (mTBI), is associated with increased risk of neuronal damage and neurodegeneration, leading to dementia, in particular Alzheimer’s disease (AD). The tell-tale histopathological defects of AD, deposits of amyloid and protein tau are observed following TBI. However, little is known about the mechanisms underlying the association, and its impact on synaptic plasticity.Objectives:
This study aimed to analyze whether mTBI alters or accelerates relevant changes in synaptic plasticity at early stages of tau pathology in a mouse model.Methods:
A total of 24 mice were analyzed in this study, comprising Tau.P301L transgenic mice and age and background matched wild-type mice as controls. Animals received a mild single-dose closed-head impact injury or a sham surgery. We measured ex vivo parameters of basal synaptic excitability, short-term synaptic plasticity and long-term potentiation.Results:
While no changes in basal synaptic excitability and presynaptic short-term plasticity were observed, long-term potentiation (LTP) in the CA1 region was severely impaired. This deficit was aggravated in the Tau.P301L mice compared to wild-type control animals.Conclusions:
Our data imply a high health risk of even a single mTBI episode. Single-impact mTBI combined with genetic predisposition is proposed to trigger signals implicated in neurodegeneration.Keywords
Mild Traumatic Brain Injury Neurodegeneration Synaptic Plasticity LTP
1. Background
Traumatic brain injury (TBI) is a common and often devastating health issue. Among the many forms of TBI, mild traumatic brain injury (mTBI) or concussive brain injury constitutes the most common form (1). Thus, mTBI affects more people and occurs more frequently than the more severe forms of TBI. Mild traumatic brain injury has been associated with, or causes diffuse neuronal damage, apoptosis and metabolic changes (2, 3). Although 80%-90% of all mTBI effects are resolved spontaneously within a couple of weeks, some functional ailments can persist for months (4). Axonal injury has been suggested to be a primary factor of adverse outcomes following TBI (5). Furthermore, TBI has been proposed to be a risk and initiating factor for the later development of neurodegenerative diseases, including Alzheimer’s disease (AD) and chronic traumatic encephalopathy (CTE) (1). Several studies observed a history of TBI to be the strongest epigenetic risk factor for neurodegenerative diseases (6-9). The diagnostic histopathological defects of AD are amyloid plaques and neurofibrillary threads and tangles (NFT) composed of hyperphosphorylated protein tau (10). Protein tau is a cytoplasmic, microtubule-associated protein (MAP) that is normally mainly present or enriched in axons.
Many neurodegenerative diseases are caused by aggregation of protein tau in different brain regions and types of neurons without any indications of amyloid plaque deposition, and are commonly designated as tauopathies (11-14). Various mutations in the tau-encoding gene cause subtypes of frontotemporal dementia clinically similar to AD, which itself is a secondary tauopathy. In AD, tau pathology but not amyloid deposition correlates with disease severity and cognitive decline (15). Tau pathology has been documented in brain of humans after severe TBI and in boxers who sustained a number of concussions resulting in CTE, eventually resulting in dementia pugilistica (16-18). Open-skull TBI accelerated tau pathology in young 3xTg-AD and Tau.P301L mice, resembling human tauopathy in both axonal and somatodendritic compartments (19). The posttraumatic tau pathology seemed to be independent of amyloid pathology. Furthermore, closed-skull mTBI in APP/PS1 knock-in mice led to greater cognitive impairment via mechanisms that involved neuroinflammatory responses mediated by glia, while eventual tau-mediated pathology was not investigated (20). However, it remains unclear whether and how closed-skull mTBI affects hippocampal synaptic plasticity, a sensitive marker of synaptic pathology, in a tauopathy model (20-22). Therefore, we examined in Tau.P301L mice that model tauopathy associated with fronto-temporal dementia and AD (23) whether mTBI induced by closed-head injury (24) led to chronic changes in hippocampal synaptic plasticity.
2. Objectives
The aim of this study was to define the impact of mTBI in a mouse model to probe whether TBI alters or accelerates relevant changes in synaptic plasticity at an early stage of tau pathology.
3. Methods
3.1. Animals
Tau.P301L transgenic mice in the FVB/N genetic background express the longest human tau isoform with the P301L mutation (tau-4R/2N-P301L) under control of the mouse thy1 gene promoter (23, 25). Male and female mice used in the present study were heterozygous for the transgene. All transgenic mice were genotyped by standard polymerase chain reaction (PCR) methods on DNA extracted from tail biopsies. Wild type FVB/N mice served as controls (WT). A total of 24 mice, aged 8-10 weeks at the time of surgery, were randomized into 4 groups: FVB/Sham control group (n = 6) and FVB/mTBI group (n = 6), Tau.P301L/Sham (n = 5) and Tau.P301L/mTBI group (n = 7). All animals were kept in standard animal cages under conventional laboratory conditions (12 h/12 h light-dark cycle, 22°C), with ad libitum access to food and water. Animals were maintained and experiments were conducted in accordance with university regulations and the European Community Council Directive (86/609/EC).
3.2. Surgery
Experimental mild traumatic brain injury was induced using the concussive closed head trauma device by Flierl et al. (24). Surgery was performed under anesthesia by chloral hydrate (400 mg/kg intraperitoneally), with analgesia provided by injection of buprenorphine (0.1 mg/kg subcutaneously). Additionally, local anesthesia over the incision site was provided by local application of lidocaine. A mid-line longitudinal incision exposed the skull. Mild traumatic brain injury was enforced with a metal rod weighing 333 g, dropped from a height of 1.5 cm onto the skull over the sagittal suture, anterior to the lambdoid suture and posterior to the coronal suture. The rod was retracted immediately to prevent an unwarranted secondary impact. Surgical sutures were used to seal the skin immediately after the impact. Sham-operated control mice were subjected to anesthesia, analgesia, scalp incision and suturing only.
3.3. Electrophysiological Recordings
Long-term electrophysiological recordings were performed as reported previously (26, 27). In brief, mice were killed by cervical dislocation and their hippocampus was rapidly dissected into ice-cold artificial cerebrospinal fluid (ACSF), saturated with carbogen (95% O2, 5% CO2). The composition of ACSF was (in mM) 124 NaCl, 4.9 KCl, 24.6 NaHCO3, 1.20 KH2PO4, 2.0 CaCl2, 2.0 MgSO4, and 10.0 glucose (pH 7.4). Transverse slices (400 µm thick) were prepared from the dorsal area of the hippocampus with a tissue chopper and placed into a submerged-type chamber, kept at 33°C and continuously perfused with ACSF (flow-rate 2.5 mL/min). After recovery for 90 min, two slices were selected and tungsten electrodes were placed in the stratum radiatum of area CA1 of each slice. For recording of field excitatory postsynaptic potentials (fEPSPs), glass electrodes (filled with ACSF, 3 - 7MΩ) were lowered into the stratum radiatum about 200 µm apart from the stimulation electrodes. The time course of the field EPSP was measured as the descending slope function for all sets of experiments. After input/output curves had been established, the stimulation strength was adjusted to elicit a fEPSP-slope of 35% of the maximum and kept constant throughout the experiment. Paired-pulse facilitation (PPF) was investigated by applying two pulses in rapid succession (interpulse intervals of 10, 20, 50, 100, 200 and 500 ms, respectively) at 120 s intervals. During baseline recording, three single stimuli (0.1 ms pulse-width; 10 s interval) were measured every 5 min and averaged. For LTP induction, a single theta-burst stimulation (TBS, 10 bursts of four stimuli at 100 Hz, applied every 200 ms; pulse width, 0.2 ms) was applied. Immediately after TBS, evoked responses were monitored at 1, 4, 7 and 10 min and then subsequently every 5 minutes until the end of the recordings at 120 minutes. Experiments of sham operated and mTBI, transgenic and WT mice were interleaved with each other. LTP was recorded five weeks postinjury.
3.4. Statistics
Differences between groups were examined using unpaired student’s t-test or repeated measures analysis of variance (ANOVA). Greenhouse-Geisser correction was used if violations of the sphericity assumption in the ANOVAs were indicated. For a further analysis of interaction effects, simple effect analyses and Bonferroni adjusted post hoc pairwise comparisons were conducted. For within-group comparisons, Wilcoxon matched-pairs signed rank test was applied. All data are presented as mean ± SEM, where n refers to the number of animals tested.
4. Results
4.1. mTBI Effects on Hippocampal Short- and Long-Term Plasticity
Because the CA1-region of the hippocampus is in particular vulnerable to various functional disturbances like ischemia, and neurodegenerative diseases, including AD-related pathology (28, 29) we analyzed hippocampal slices and recorded field EPSPs (fEPSPs) in this region. First, we measured the input-output properties and observed no differences between sham and mTBI mice, or a contribution of the transgene, in fEPSP slopes regardless of stimulus strength, F < 1 (Figure 1 and 2A). Likewise, there was no effect of mTBI or transgene when short-term plasticity was inspected by delivering two pulses at different interstimulus intervals in rapid succession (paired-pulse measurements, F < 1.0; Figure 1 and 2B).
Electrophysiological Examination of Basic Excitability, Short-Term Plasticity and Long-Term Potentiation (LTP) in the CA1 Region of Wild-Type Control Mice in Response to mTBI and Sham Operation, Respectively
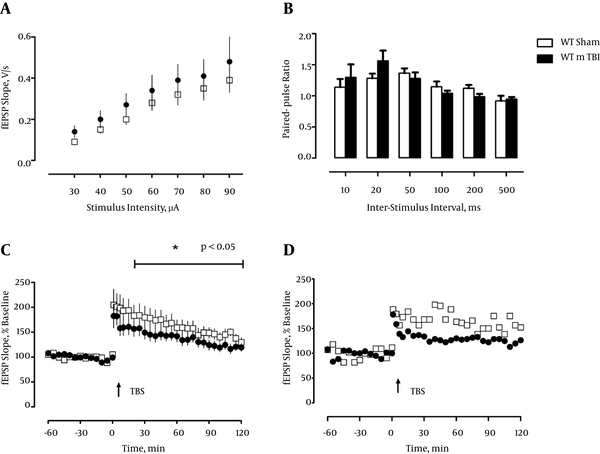
Electrophysiological Examination of Basic Excitability, Short-Term Plasticity and LTP in the CA1 Region of Tau.P301L Mice in Response to mTBI and Sham Operation
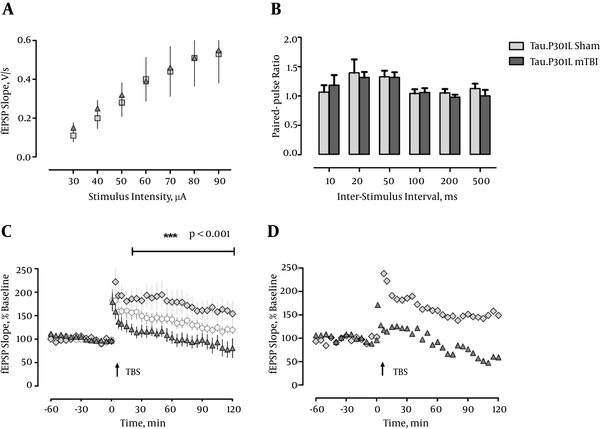
Next we induced an unsaturated, weak type of LTP by a single train of theta-burst stimulation (TBS), which we had demonstrated previously to be very sensitive to disturbances in the synaptic circuitry in the hippocampus (27, 30). Induction of LTP in slices from sham-operated WT animals and Tau.P301L mice, five weeks post surgery resulted in pronounced, robust LTP that was retained until the end of recording (120 minutes WT: 176.298 ± 16.659%; Tau.P301L: 181.401 ± 16.649%). In contrast, mTBI resulted in a significantly lowered and more decremental potentiation, which was most significant in the Tau.P301L mice, which developed only short-term potentiation, which returned to baseline after about 25 min (see Figure 2C). The potentiation in the WT mTBI group was longer maintained and returned to baseline only after about 95 minutes (see Figure 1C). Statistical analysis by repeated measure ANOVA confirmed the significant effect (F (3, 42) = 4.849, P < 0.01, ηp2 = 0.257). We concluded that our results clearly demonstrated the detrimental effect of mTBI on CA1-LTP in all mice, which was significantly aggravated by the tau pathology in the Tau.P301L mice.
5. Discussion
Closed-skull mild traumatic brain injury has been associated with long-term cognitive deficits (31-33) and diffuse cellular damage and apoptosis (3, 34). Even mild mechanical trauma to the head can initiate a complex cascade of neurochemical and neurometabolic events. Traumatic brain injury has been linked to the later development of a number of neurodegenerative features that are typical of diseases like CTE and AD (1). Molecular mechanisms of mTBI and CTE are still only poorly understood and as a consequence, there are no current pharmacological treatments that prevent or considerably reduce cognitive impairments associated with mTBI and CTE in humans (35). Diseases like CTE and AD converge mechanistically onto synaptic dysfunctions, termed synaptopathies (36). We therefore aimed in this study to probe whether TBI altered or accelerated dementia-related changes in synaptic plasticity. These were reported to be prone to synaptic disturbances (20-23, 29, 30, 37-40).
We applied a model of mild single, closed-skull TBI onto Tau.P301L mice and their wild-type controls, matched for age, gender and genetic background. In general, human mutant Tau.P301L is pathogenic and critical for cognitive decline in older mice (23, 41), while cognition in very young (1 - 2 month) Tau.P301L mice was improved compared to wild-type controls (41). Furthermore, ex-vivo LTP measured in the dentate gyrus in the hippocampus was improved in adult Tau.P301L mice compared to FVB wild-type controls (42). We observed that the heterozygous expression of human Tau.P301L is sufficient to cause impaired cognition and behavior in 16 - 20 week old animals (data not shown). For these reasons we analyzed heterozygous Tau.P301L mice and tested whether the combination of a single episode of mTBI with weak tau-related pathology was sufficient to trigger lasting chances of synaptic plasticity commonly associated with AD or CTE.
Interestingly, we observed that a mild, closed-skull impact resulted in pronounced impairments of LTP in WT and to an even greater extent in tau transgenic mice. Because basal synaptic transmission and presynaptic short-term plasticity were not altered by mTBI in WT and tau transgenic mice, the inability to induce LTP does not seem to be caused by a general disturbance of synaptic transmission or presynaptic functioning but rather by a specific LTP-deficit. Importantly, our results indicate that already a few weeks after a single mTBI incident, an acceleration of specific disturbances in the LTP machinery was evident. The disturbance is typically associated with an age-related tau-driven phenotype and manifests itself as an impairment of LTP maintenance. Given that deficits in LTP correlate with impaired cognitive performance (43), our data imply a high health risk of even a single mTBI episode. Single-impact mTBI combined with genetic predisposition might in itself be sufficient to trigger signaling events implicated in CTE. Repeated mTBI that occurs for example in athletes and military personnel is then proposed to aggravate the pathological mechanisms even further.
References
-
1.
Dashnaw ML, Petraglia AL, Bailes JE. An overview of the basic science of concussion and subconcussion: where we are and where we are going. Neurosurg Focus. 2012;33(6):1-9. [PubMed ID: 23199428]. https://doi.org/10.3171/2012.10.FOCUS12284.
-
2.
Barkhoudarian G, Hovda DA, Giza CC. The molecular pathophysiology of concussive brain injury. Clin Sports Med. 2011;30(1):33-48. [PubMed ID: 21074080]. https://doi.org/10.1016/j.csm.2010.09.001.
-
3.
Tashlykov V, Katz Y, Gazit V, Zohar O, Schreiber S, Pick CG. Apoptotic changes in the cortex and hippocampus following minimal brain trauma in mice. Brain Res. 2007;1130(1):197-205. [PubMed ID: 17174280]. https://doi.org/10.1016/j.brainres.2006.10.032.
-
4.
McCrea M, Guskiewicz KM, Marshall SW, Barr W, Randolph C, Cantu RC, et al. Acute effects and recovery time following concussion in collegiate football players. Br J Sports Med. 2004;38:369-71. [PubMed ID: 14625332]. https://doi.org/10.1001/jama.290.19.2556.
-
5.
Smith DH, Meaney DF, Shull WH. Diffuse axonal injury in head trauma. J Head Trauma Rehabil. 2003;18(4):307-16. [PubMed ID: 16222127].
-
6.
Chauhan NB. Chronic neurodegenerative consequences of traumatic brain injury. Restor Neurol Neurosci. 2014;32(2):337-65. [PubMed ID: 24398724]. https://doi.org/10.3233/RNN-130354.
-
7.
Mortimer JA, Van Duijn CM, Chandra V, Fratiglioni L, Graves AB, Heyman A, et al. Head trauma as a risk factor for Alzheimer's disease: a collaborative re-analysis of case-control studies. Int J Epidemiol. 1991;20(2):28-S35.
-
8.
Sivanandam TM, Thakur MK. Traumatic brain injury: a risk factor for Alzheimer's disease. Neurosci Biobehav Rev. 2012;36(5):1376-81. [PubMed ID: 22390915]. https://doi.org/10.1016/j.neubiorev.2012.02.013.
-
9.
Fleminger S, Oliver DL, Lovestone S, Rabe-Hesketh S, Giora A. Head injury as a risk factor for Alzheimer's disease: the evidence 10 years on; a partial replication. J Neurol Neurosurg Psychiatry. 2003;74(7):857-62. [PubMed ID: 12810767].
-
10.
Gotz J, Ittner A, Ittner LM. Tau-targeted treatment strategies in Alzheimer's disease. Br J Pharmacol. 2012;165(5):1246-59. [PubMed ID: 22044248]. https://doi.org/10.1111/j.1476-5381.2011.01713.x.
-
11.
Delacourte A, Buee L. Tau pathology: a marker of neurodegenerative disorders. Curr Opin Neurol. 2000;13(4):371-6. [PubMed ID: 10970052].
-
12.
Duyckaerts C. Tau pathology in children and young adults: can you still be unconditionally baptist? Acta Neuropathol. 2011;121(2):145-7. [PubMed ID: 21225273]. https://doi.org/10.1007/s00401-010-0794-7.
-
13.
Ingram EM, Spillantini MG. Tau gene mutations: dissecting the pathogenesis of FTDP-17. Trends Mol Med. 2002;8(12):555-62. [PubMed ID: 12470988].
-
14.
Ludolph AC, Kassubek J, Landwehrmeyer BG, Mandelkow E, Mandelkow EM, Burn DJ, et al. Tauopathies with parkinsonism: clinical spectrum, neuropathologic basis, biological markers, and treatment options. Eur J Neurol. 2009;16(3):297-309. [PubMed ID: 19364361]. https://doi.org/10.1111/j.1468-1331.2008.02513.x.
-
15.
Braak H, Braak E. Staging of Alzheimer's disease-related neurofibrillary changes. Neurobiol Aging. 1995;16(3):271-8. [PubMed ID: 7566337].
-
16.
Ikonomovic MD, Uryu K, Abrahamson EE, Ciallella JR, Trojanowski JQ, Lee VM, et al. Alzheimer's pathology in human temporal cortex surgically excised after severe brain injury. Exp Neurol. 2004;190(1):192-203. [PubMed ID: 15473992]. https://doi.org/10.1016/j.expneurol.2004.06.011.
-
17.
Johnson VE, Stewart W, Smith DH. Widespread tau and amyloid-beta pathology many years after a single traumatic brain injury in humans. Brain Pathol. 2012;22(2):142-9. [PubMed ID: 21714827]. https://doi.org/10.1111/j.1750-3639.2011.00513.x.
-
18.
Uryu K, Chen XH, Martinez D, Browne KD, Johnson VE, Graham DI, et al. Multiple proteins implicated in neurodegenerative diseases accumulate in axons after brain trauma in humans. Exp Neurol. 2007;208(2):185-92. [PubMed ID: 17826768]. https://doi.org/10.1016/j.expneurol.2007.06.018.
-
19.
Tran HT, LaFerla FM, Holtzman DM, Brody DL. Controlled cortical impact traumatic brain injury in 3xTg-AD mice causes acute intra-axonal amyloid-beta accumulation and independently accelerates the development of tau abnormalities. J Neurosci. 2011;31(26):9513-25. [PubMed ID: 21715616]. https://doi.org/10.1523/JNEUROSCI.0858-11.2011.
-
20.
Selkoe DJ. Alzheimer's disease is a synaptic failure. Science. 2002;298(5594):789-91. [PubMed ID: 12399581]. https://doi.org/10.1126/science.1074069.
-
21.
Marchetti C, Marie H. Hippocampal synaptic plasticity in Alzheimer's disease: what have we learned so far from transgenic models? Rev Neurosci. 2011;22(4):373-402. [PubMed ID: 21732714]. https://doi.org/10.1515/RNS.2011.035.
-
22.
Rowan MJ, Klyubin I, Cullen WK, Anwyl R. Synaptic plasticity in animal models of early Alzheimer's disease. Philos Trans R Soc Lond B Biol Sci. 2003;358(1432):821-8. [PubMed ID: 12740129]. https://doi.org/10.1098/rstb.2002.1240.
-
23.
Terwel D, Lasrado R, Snauwaert J, Vandeweert E, Van Haesendonck C, Borghgraef P, et al. Changed conformation of mutant Tau-P301L underlies the moribund tauopathy, absent in progressive, nonlethal axonopathy of Tau-4R/2N transgenic mice. J Biol Chem. 2005;280(5):3963-73. [PubMed ID: 15509565]. https://doi.org/10.1074/jbc.M409876200.
-
24.
Flierl MA, Stahel PF, Beauchamp KM, Morgan SJ, Smith WR, Shohami E. Mouse closed head injury model induced by a weight-drop device. Nat Protoc. 2009;4(9):1328-37. [PubMed ID: 19713954]. https://doi.org/10.1038/nprot.2009.148.
-
25.
Terwel D, Muyllaert D, Dewachter I, Borghgraef P, Croes S, Devijver H, et al. Amyloid activates GSK-3beta to aggravate neuronal tauopathy in bigenic mice. Am J Pathol. 2008;172(3):786-98. [PubMed ID: 18258852]. https://doi.org/10.2353/ajpath.2008.070904.
-
26.
Ahmed T, Sabanov V, D'Hooge R, Balschun D. An N-methyl-D-aspartate-receptor dependent, late-phase long-term depression in middle-aged mice identifies no GluN2-subunit bias. Neuroscience. 2011;185:27-38. [PubMed ID: 21504782]. https://doi.org/10.1016/j.neuroscience.2011.04.006.
-
27.
Denayer E, Ahmed T, Brems H, Van Woerden G, Borgesius NZ, Callaerts-Vegh Z, et al. Spred1 is required for synaptic plasticity and hippocampus-dependent learning. J Neurosci. 2008;28(53):14443-9. [PubMed ID: 19118178]. https://doi.org/10.1523/JNEUROSCI.4698-08.2008.
-
28.
Crepel V, Epsztein J, Ben-Ari Y. Ischemia induces short- and long-term remodeling of synaptic activity in the hippocampus. J Cell Mol Med. 2003;7(4):401-7. [PubMed ID: 14754508].
-
29.
Oddo S, Caccamo A, Shepherd JD, Murphy MP, Golde TE, Kayed R, et al. Triple-transgenic model of Alzheimer's disease with plaques and tangles: intracellular Abeta and synaptic dysfunction. Neuron. 2003;39(3):409-21. [PubMed ID: 12895417].
-
30.
Wilsch VW, Behnisch T, Jager T, Reymann KG, Balschun D. When are class I metabotropic glutamate receptors necessary for long-term potentiation? J Neurosci. 1998;18(16):6071-80. [PubMed ID: 9698302].
-
31.
Luo J, Nguyen A, Villeda S, Zhang H, Ding Z, Lindsey D, et al. Long-term cognitive impairments and pathological alterations in a mouse model of repetitive mild traumatic brain injury. Front Neurol. 2014;5:12. [PubMed ID: 24550885]. https://doi.org/10.3389/fneur.2014.00012.
-
32.
Petraglia AL, Plog BA, Dayawansa S, Chen M, Dashnaw ML, Czerniecka K, et al. The spectrum of neurobehavioral sequelae after repetitive mild traumatic brain injury: a novel mouse model of chronic traumatic encephalopathy. J Neurotrauma. 2014;31(13):1211-24. [PubMed ID: 24766454]. https://doi.org/10.1089/neu.2013.3255.
-
33.
Yang SH, Gustafson J, Gangidine M, Stepien D, Schuster R, Pritts TA, et al. A murine model of mild traumatic brain injury exhibiting cognitive and motor deficits. J Surg Res. 2013;184(2):981-8. [PubMed ID: 23622728]. https://doi.org/10.1016/j.jss.2013.03.075.
-
34.
Tweedie D, Milman A, Holloway HW, Li Y, Harvey BK, Shen H, et al. Apoptotic and behavioral sequelae of mild brain trauma in mice. J Neurosci Res. 2007;85(4):805-15. [PubMed ID: 17243171]. https://doi.org/10.1002/jnr.21160.
-
35.
Aungst SL, Kabadi SV, Thompson SM, Stoica BA, Faden AI. Repeated mild traumatic brain injury causes chronic neuroinflammation, changes in hippocampal synaptic plasticity, and associated cognitive deficits. J Cereb Blood Flow Metab. 2014;34(7):1223-32. [PubMed ID: 24756076]. https://doi.org/10.1038/jcbfm.2014.75.
-
36.
Grant SG. Synaptopathies: diseases of the synaptome. Curr Opin Neurobiol. 2012;22(3):522-9. [PubMed ID: 22409856]. https://doi.org/10.1016/j.conb.2012.02.002.
-
37.
Ahmed T, Blum D, Burnouf S, Demeyer D, Buee-Scherrer V, D'Hooge R, et al. Rescue of impaired late-phase long-term depression in a tau transgenic mouse model. Neurobiol Aging. 2015;36(2):730-9. [PubMed ID: 25443285]. https://doi.org/10.1016/j.neurobiolaging.2014.09.015.
-
38.
LaFerla FM, Oddo S. Alzheimer's disease: Abeta, tau and synaptic dysfunction. Trends Mol Med. 2005;11(4):170-6. [PubMed ID: 15823755]. https://doi.org/10.1016/j.molmed.2005.02.009.
-
39.
Rosenmann H, Grigoriadis N, Eldar-Levy H, Avital A, Rozenstein L, Touloumi O, et al. A novel transgenic mouse expressing double mutant tau driven by its natural promoter exhibits tauopathy characteristics. Exp Neurol. 2008;212(1):71-84. [PubMed ID: 18490011]. https://doi.org/10.1016/j.expneurol.2008.03.007.
-
40.
Van der Jeugd A, Hochgrafe K, Ahmed T, Decker JM, Sydow A, Hofmann A, et al. Cognitive defects are reversible in inducible mice expressing pro-aggregant full-length human Tau. Acta Neuropathol. 2012;123(6):787-805. [PubMed ID: 22532069]. https://doi.org/10.1007/s00401-012-0987-3.
-
41.
Kremer A, Maurin H, Demedts D, Devijver H, Borghgraef P, Van Leuven F. Early improved and late defective cognition is reflected by dendritic spines in Tau.P301L mice. J Neurosci. 2011;31(49):18036-47. [PubMed ID: 22159117]. https://doi.org/10.1523/JNEUROSCI.4859-11.2011.
-
42.
Boekhoorn K, Terwel D, Biemans B, Borghgraef P, Wiegert O, Ramakers GJ, et al. Improved long-term potentiation and memory in young tau-P301L transgenic mice before onset of hyperphosphorylation and tauopathy. J Neurosci. 2006;26(13):3514-23. [PubMed ID: 16571759]. https://doi.org/10.1523/JNEUROSCI.5425-05.2006.
-
43.
Citri A, Malenka RC. Synaptic plasticity: multiple forms, functions, and mechanisms. Neuropsychopharmacology. 2008;33(1):18-41. [PubMed ID: 17728696]. https://doi.org/10.1038/sj.npp.1301559.