Abstract
Objectives:
To explore the value of T2 and T1 mapping in the early detection of degenerating articular cartilage.Materials and Methods:
Twenty-six New Zealand rabbits were randomly divided into three groups. Groups A and B (n = 10 each) had the right knee joint injected with either collagenase type II or papain. The equivalent dilution was injected into the left knee joint as control. In addition, six rabbits served as blank group. Both knees were scanned using MRI, and T2 and T1 values of the cartilage were measured. The femurs were examined microscopically and immunohistologically. T2 and T1 values were compared within and between groups.Results:
In group A, immunohistochemistry found that collagen type II decreased in the treated knees. T2 and T1 values were higher in the treated knees than the controls (P < 0.001). In group B, Alcian blue staining showed reduced proteoglycan in the treated knees. The T1 values in the treated knees were higher than the controls (P < 0.001).Conclusion:
T2 and T1 mapping may be useful in the early diagnosis of cartilage degeneration. The changes in collagen content of the cartilage were related to T2 relaxation time; whereas, T1 relaxation time was affected by both collagen and proteoglycan.Keywords
Osteoarthritis Magnetic Resonance Imaging T2 Mapping T1 Mapping Collagenase Type II Papain
1. Background
Osteoarthritis (OA) is a common, noninflammatory disease. Traditionally, it was considered a disease of articular cartilage. The current concept holds that osteoarthritis involves the entire joint organ, including the subchondral bone, menisci, ligaments, periarticular muscle, capsule, and synovium. The signature pathologic feature of OA is hyaline articular cartilage loss, which is loss of cartilage matrix (proteoglycan and collagen type II mainly) (1). Currently, there is no cure for OA, and despite treatment, affected individuals often continue to experience pain and decreased quality of life. As such, prevention and early detection/diagnosis are important to potentially delay disease progression. This study established models of knee OA in rabbits induced by two different proteases and explored changes in the magnetic resonance imaging (MRI) manifestations of knee joint after proteoglycan/collagen type II loss. The MRI findings were compared with the pathologic results to determine if MRI was a suitable method for evaluating articular cartilage lesions and identifying the predominant type of cartilage matrix lost in OA. These results may help improve the diagnosis and treatment of OA and potentially provide an alternate technique to monitor treatment efficacy.
2. Materials and Methods
2.1. Equipment, Subject Stratification, and Establishing the OA Models
In accordance with the recommendations of the Weatherall report, “The use of non-human primates in research”, and approved by animal care and use committee of Jinan University, 26 New Zealand white rabbits were randomly divided into three groups. Rabbits in group A (n = 10) were injected with 0.5 ml (2 mg) of 0.4% collagenase type II (Sigma Co., St. Louis, MO, USA) solution into the cavity of the right knee joint. The injection was repeated once 3 days later. The left knee joint served as the control and was injected with 0.5 mL sterile phosphate buffered saline (pH 7.4) without type II collagenase. Model construction was completed 4 weeks after the last injection. Rabbits in group B were injected with 0.5 mL (8 mg) of 1.6% papain (Sigma Co., St. Louis, MO, USA) solution into the cavity of the right knee joint. The injection was repeated once on day 4. The left knee joint served as the control, and 0.5 ml sterile phosphate buffered saline (pH 7.4) without papain was injected into the joint cavity. Model construction was completed 6 weeks after the last injection. Rabbits in group C did not receive any injection and served as the blank control group.
2.2. MRI Examination and Articular Cartilage Analysis
Rabbits included in groups A and B underwent MRI within 24 hours after completion of model construction (weeks 4 and 6, respectively). Three rabbits in group C underwent MRI at the same time as the rabbits in group A, and the remaining three rabbits underwent MRI at the same time as the rabbits in group B.
An Echospeed plus Signa MR/i 1.5 T (GE MedicalSystems, Milwaukee, WI) MRI, and 3 inch of coil were used. Sodium pentobarbital solution (3%) was injected via the marginal ear vein (1 mL/kg) to induce anesthesia. After the rabbit was anesthetized, both knees were fixed and sagittal T2 weighted imaging (T2WI), three-dimensional fat-suppressed spoiled gradient-recalled echo (3D-FS-SPGR), T2 mapping, and T1 mapping were performed, first on the left knee then the right knee. Scanning parameters: T2WI(repetition time (TR)/echo time (TE) -2000/85), thickness 3 mm, increment 0.5 mm, field of view 12 × 12 cm, matrix 320 × 224, pulse three times; 3D FS-SPGR(TR/TE -40/8, flip angle 12°), thickness 2.4 mm, field of view 14 × 14 cm, matrix 256 × 192, pulse two times; T2mapping (TE 17 ms, 34 ms, 51 ms, 68 ms, TR 1060 ms), thickness 2 mm, increment 0.5 mm, field of view 12 × 12 cm, matrix 256 × 160, pulse two times; T1mapping(TE 20 ms, TR 100 ms, 200 ms, 300 ms, 400 ms, 500 ms, 800 ms, 1000 ms), thickness 2mm, increment 0.5 mm, field of view 12 × 12 cm, matrix 256 × 192, pulse four times.
The GE Sun Advantage Workstation 4.2 photo working station and related post-processing software were used for data analysis. Both T2 and T1 values of cartilages were measured. Three distinct regions in the anterior, middle, and posterior lateral femoral condyle were selected for T2 and T1 measurements, and the average was calculated. Readings were made by two experienced musculoskeletal radiologists who had been working over 15 years.
2.3. Rabbit Articular Cartilage Sample Disposal
All rabbits were sacrificed immediately after imaging via air embolism. The knee joints of all rabbits were completely removed and soaked in 10% formalin solution. The samples were decalcified with hydrochloric acid, and a 0.5 cm thick tissue block was collected from the lateral femur condyle (in the sagittal plane, in the same direction as the MRI). The tissue blocks were embedded in paraffin, sectioned, and stained with hematoxylin and eosin. Changes in proteoglycan content were analyzed by Alcian blue staining. Collagen type II immunohistochemistry (COL2A1, Santa Cruz Biotechnology, Inc., Santa Cruz, CA, USA; SP-test) to assess type II collagen content was also performed.
2.4. Statistical Analysis
All data were analyzed using SPSS (Statistical Product and Service Solutions) Released 2007. SPSS for Windows, Version 16.0. Chicago, SPSS Inc statistical software. ANOVA (Analysis of variance) of the data was carried out to examine the differences in T2 and T1 values of the control cartilage samples from all three groups to determine whether the diluent had any effect on the T2 and T1 values. The data were in normal distribution (P > 0.05). A paired two-sample t-test was then carried out to compare the T2 and T1 values measured in the treated knees and control knees of rabbits in both groups A and B. Finally, independent sample t-test was performed to compare the T2 and T1 values measured in only the treated knees of rabbits in groups A and B. A P < 0.05 was considered statistically significant.
3. Results
In the T2WI sequence, the articular cartilage showed either isointense or hyperintense signals compared to muscles. In the 3D FS SPGR sequence, the cartilage had obvious hyperintense signals. Conventional MR sequences (T2WI, 3D FS SPGR) did not identify any obvious differences in the articular cartilage between the three groups.
ANOVA of cartilage relaxation time on the control knee in each of the three groups showed that the mean T2 and T1 values were not significantly different, except for the impact of diluents. Table 1 shows the comparisons of T2 and T1 values between group A and B.
Comparison of T2 and T1 Values Between Group A and B (n = 10)a
Variables | Group A | Group B | ||
---|---|---|---|---|
T2 value, ms | T1 value, ms | T2 value, ms | T1 value, ms | |
Treated side | 48.10 ± 3.92 | 1041.3 ± 81.9 | 35.69 ± 1.77 | 992.6 ± 65.5 |
Control side | 35.68 ± 1.57 | 694.4 ± 43.9 | 36.37 ± 1.69 | 689.3 ± 53.0 |
Paired sample t-test showed that the T2 (t = 10.294, P < 0.001) and T1 values (t = 18.17, P < 0.001) of the cartilage on the lateral femoral condyle between the treated and control knees were different in group A. The T1 and T2 relaxation time of the cartilage in the treated knee was higher than normal. T2 mapping (Figure 1) showed that normal articular cartilage was uniform light green in color, but the cartilage in the treated knee was more yellow in color than the control knee. Similarly, T1 mapping (Figure 2) showed that normal articular cartilage had a uniform green color, but the cartilage in the treated knee was more yellow in color than the control side, and the signals were less uniform.
Group A (collagenase type II injected in the right knee). T2 mapping shows that normal articular cartilage (A) is uniform light green in color, but the cartilage in the treated knee (B) is more yellow in color than the control knee, and the signals are less uniform.
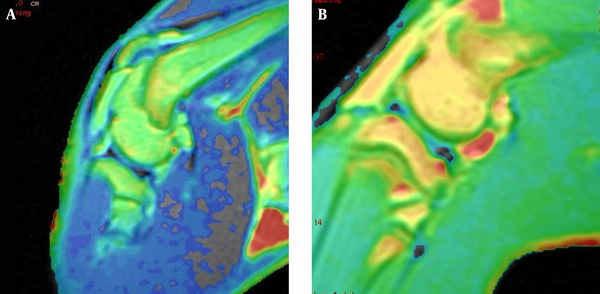
Group A (collagenase type II injected in the right knee). T1 mapping shows that normal articular cartilage (A) has a uniform green color, but the cartilage in the treated knee (B) is more yellow in color than the control side, and the signals are less uniform.
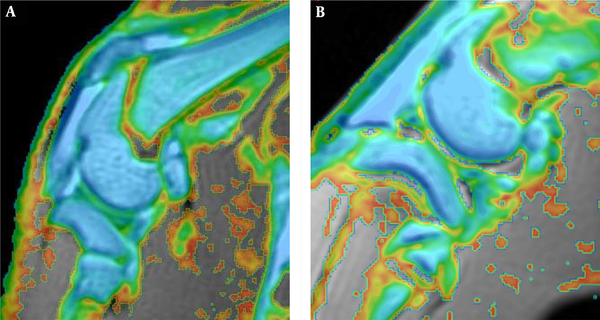
Histologically (Figure 3), routine hematoxylin and eosin staining revealed a rough cartilage surface in the treated knee and formation of gap junctions. Some cartilage surfaces had fibril formation, and the number of chondrocytes increased. Necrosis, tufted proliferation, hyperplasia, disordered cell arrangement, and disappearance of normal cartilage cell alignment were also noted. Alcian blue staining revealed no reduction in proteoglycan in the treated knees compared to the control knees. Immunohistochemistry found that collagen type II in the treated knees significantly decreased compared with the control knees. The superficial surface layer and transitional layer of the articular cartilage had the most pronounced decrease in collagen type II. Further, the collagen network was ruptured and destroyed.
A, Group A control knee shows the basic structure of the normal cartilage. (H&E × 100); B, Group A treated knee shows the basic structure of the abnormal cartilage. (H&E×100); C, Group A control knee shows proteoglycan of the normal cartilage. (Alcian blue staining × 100); D, Group A treated knee shows proteoglycan of the abnormal cartilage. (Alcian blue staining × 100); E, Group A control knee shows collagen type II of the normal cartilage. (Immunohistochemistry × 100); F, Group A treated 1 knee shows collagen type II of the abnormal cartilage (Immunohistochemistry × 100).
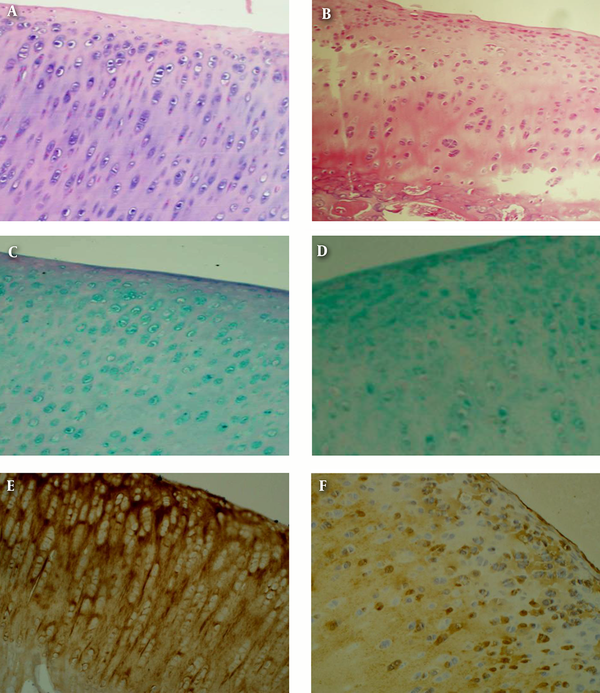
The T1 value (t = 18.17, P < 0.001) of the cartilage on the lateral femoral condyle in the treated and control knees was different in group B. The T1 relaxation time of the cartilage in the treated knees was higher than normal, but the T2 value was not significantly different (t = 0.982, P = 0.353). T2 mapping (Figure 4) showed that both the normal and the treated articular cartilage had uniform light green color, and T1 mapping (Figure 5) showed that normal articular cartilage had a uniform green color, but the cartilage in the treated knees was more yellow in color than the control knees, and the signals were less uniform.
Group B (papain injected in the right knee). T2 mapping shows that both the normal (A) and the treated (B) articular cartilage have uniform light green color.
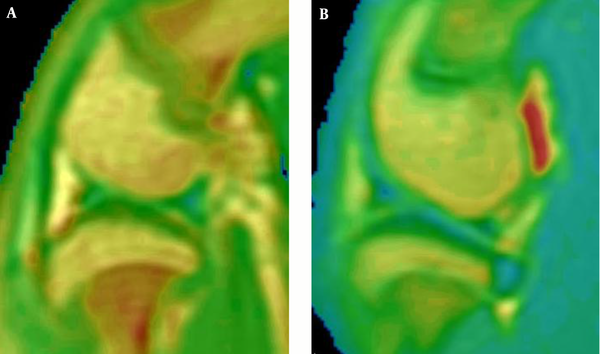
T1 mapping shows that normal articular cartilage (A) has a uniform green color, but the cartilage in the treated knees (B) are more yellow in color than the control knees, and the signals are less uniform.
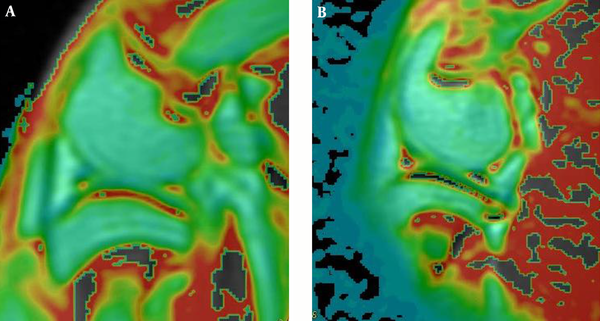
As shown in Figure 6, routine hematoxylin and eosin staining revealed a rough cartilage surface in the treated knees and a decreased number and disordered arrangement of chondrocytes. Necrosis and tufted proliferation of chondrocytes were also noted. Alcian blue staining showed reduced proteoglycan in the treated knees compared to the control knees. Immunohistochemistry revealed that the collagen content was not reduced significantly between the treated and control knees. Further, the chondrocytes in the treated knees appeared disarranged and had abnormal morphology.
A, Group B control knee shows the basic structure of the normal cartilage. (H&E × 100); B, Group B treated knee shows the basic structure of the abnormal cartilage. (H&E × 100); C, Group B control knee shows proteoglycan of the normal cartilage. (Alcian blue staining × 100); D, Group B treated knee shows proteoglycan of the abnormal cartilage. (Alcian blue staining × 100); E, Group B control knee shows collagen type II of the normal cartilage. (Immunohistochemistry × 100); F, Group B treated l knee shows collagen type II of the abnormal cartilage (Immunohistochemistry × 100).
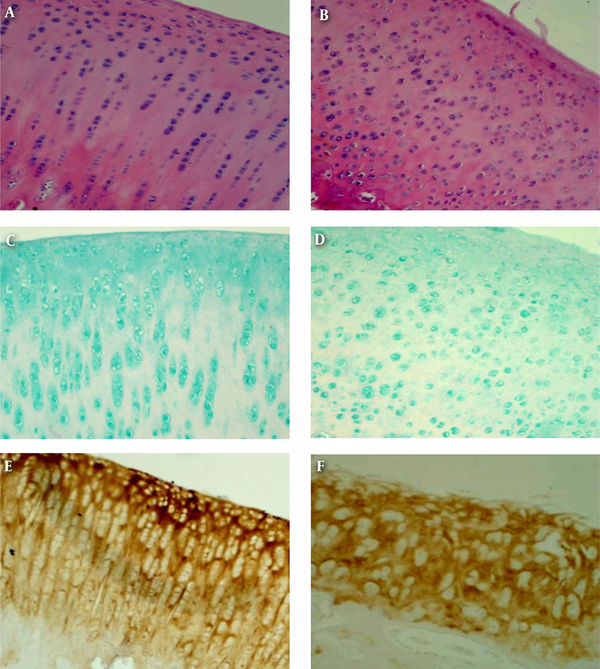
T2 values (t = 8.688, P < 0.001) of the articular cartilage on the lateral femoral condyles in the treated knees were differed between group A and B. Specifically, the T2 relaxation time in group A was greater than in group B. There was no significant difference in T1 values (t = 1.469, P = 0.160) of the articular cartilage on the lateral femoral condyles in the treated knees between groups A and B.
4. Discussion
This study used New Zealand white rabbits to construct two animal models of early OA. The anatomic structure of the rabbit knee joint is the same as the human knee joint. In addition, humans and rabbits have similar extracellular matrix components. The composition ratio of the articular cartilage is also similar, as well as the arrangement and size of the chondrocytes (2). In terms of model construction, some studies (3, 4) showed that the injection of collagenase type II or papain into the rabbit knee joint can cause rapidly progressing OA. The onset of OA is rapid with good reproducibility, and the induced OA is similar to that reported in humans. The injection of 2 mg of collagenase type II into the rabbit knee joint with a second injection on day 4 can establish a model of early OA, with predominant collagen network structural damage 4 weeks after the last injection. A model of advanced OA can be established 6 weeks after the last injection. In this study, 8 mg of papain caused significant reduction in proteoglycan in the articular cartilage 6 weeks after the last injection, without apparent damage to the collagen network.
A large number of clinical and experimental studies have confirmed that the T2 relaxation time of articular cartilage is associated with a variety of factors (5-8), including the anisotropy of the four layers of the cartilage tissue, content of collagen and water, and the direction of arrangement. Histologically, collagen fibers are arranged in parallel in the superficial layer, they are arranged randomly in the transitional layer, and vertically in the radial and calcified layers, forming a three-dimensional network of collagen fibers. Proteoglycans are scattered throughout the network. They are hydrophilic molecules that are less abundant in the superficial layer and more abundant in the radial layer (9, 10). Some researchers believe that the T2 relaxation time of articular cartilage is related to the proteoglycan content (11). The direction of arrangement of water molecules in the cartilage is parallel to that of collagen fibers. The different arrangements of collagen fibers in different layers of cartilage result in anisotropy of the distribution of water molecules, forming a stable magnetization vector angle. This is referred to as the “magic angle” effect (12), which affects the T2 relaxation time of the cartilage.
In this study, collagenase type II was used to destroy the cartilage collagen fiber network in the rabbits included in group A, and the measured T2 values were significantly higher than those measured in the control group. Papain was used to hydrolyze cartilage proteoglycan in the treated knees of rabbits in group B, and the measured T2 values were not significantly different from those measured in the control group. The authors of this report believe that the T2 relaxation time primarily reflects changes in collagen in the cartilage matrix and is not closely associated with changes in proteoglycan. After destruction of the collagen network, T2 relaxation time increased. There are several possible reasons for this increase in T2 relaxation time. First, destruction of the collagen network in group A weakened the anisotropy of the distribution of water molecules, which reduced the “magic angle” effect. As such, T2 value of the cartilage increased. Second, after destruction of collagen fibers in the superficial and transitional layers in group A, the barrier to water molecules was weakened, and the content of free water molecules significantly increased in the cartilage. The content of the corresponding hydrogen protons also increased, resulting in an increased T2 value of the cartilage. Third, after destruction of the collagen network in group A, the interactions between the water molecules and large molecules (collagen) were reduced, and the T2 values of the cartilage increased. In group B, endochondral proteoglycan was reduced; however, proteoglycan is only a small portion of the extracellular matrix. Thus, the decreased proteoglycan content only resulted in slightly reduced interactions between water molecules and macromolecules (proteoglycan) resulting in slightly increased T2 values of the cartilage. Fourth, the destruction of the collagen network in group A exposed negatively charged proteoglycans that made the proteoglycans attract more water molecules. Therefore, the water content of the articular cartilage increased, and the content of hydrogen protons increased, resulting in increased T2 values of the cartilage. In contrast, the reduction in the proteoglycan content in the cartilage in group B resulted in a slightly decreased content of the water and hydrogen protons in the articular cartilage; therefore, the T2 values of the cartilage slightly decreased. The combined actions of the aforementioned points lead to elevated T2 values in group A and insignificant change of T2 values in group B.
Cartilage damage caused by trauma occurs prior to obvious morphologic changes. The early manifestations are destruction of the ultrastructure of the collagens in the cartilage matrix and loss of collagen fibers. Those steps are followed by a decrease in the proteoglycan content, increase in amount of water, and increased T2 values of the cartilage. Then, the T2 values gradually decreased with collagen hyperplasia and repair. Therefore, T2 mapping appears to be a good tool for monitoring of the early events following trauma-induced OA. Further, T2 mapping can indirectly reflect changes in collagens in the cartilage matrix. In the early stages of OA, proteoglycan synthesis declined due to chondrocyte dysfunction, which changes the proteoglycan content. Collagens in the superficial cartilage layer degenerate first, resulting in an increase in water permeability. Next, proteoglycans progressively decrease, followed by destruction and rupture of the network structure and an increased water content. If only a reduction in proteoglycan content occurs and damage, changes to the collagen structure are not obvious so the T2 values changes are not great. With the destruction of the collagen network structure, T2 values gradually increase. The pathologic processes of early OA can be simulated at both molecular and biochemical levels to provide help for clinical diagnosis and treatment planning, as well as for assessing efficacy (e.g., collagen repair).
Most studies show that delayed gadolinium-enhanced MRI of the cartilage is a good imaging sequence for cartilage that could reflect changes in the cartilage matrix proteoglycan content through T1 mapping (T1 values) (13-15). Because glycosaminoglycans have negative charges, the contrast agent gadolinium diethylene triamine pentaacetic acid (Gd-DTPA2) enters the cartilage and concentrates at places where the glycosaminoglycan content is low. In patients with OA, if the glycosaminoglycan content decreased, the concentration of Gd-DTPA2 increased, leading to decreased T1 values of the cartilage. This method is slightly invasive and it is therefore, difficult to apply widely in clinical practice. In contrast, standard T1 mapping could be adopted to help determine whether the T1 value of cartilage is associated with changes in the biochemical composition of the cartilage.
This study shows that both OA models can lead to significantly higher cartilage T1 values than normal. The T1 values of cartilage on the control side in the collagen type II group were slightly higher than those in the papain group. That difference was not statistically significant, indicating that T1 values are more sensitive to cartilage matrix changes in early OA. Both reduction in proteoglycan and damage to the collagen network structure can cause significantly increased T1 values; however, it is still unclear what matrix change predominates. There are two possible reasons for this. First, destruction of the collagen fiber network structure and/or reduction in proteoglycan content may result in decreased interactions between large molecules in the matrix (collagen/ proteoglycans) and water molecules, resulting in enhanced free activity of water molecules and increased T1 values. Second, after destruction of the collagen fiber network structure, the content of free water molecules in the cartilage increase as well as the T1 values.
Prior to changes in cartilage morphology in early OA, changes in T1 value could be used to reflect changes in the cartilage matrix. T1 values are sensitive to changes in both proteoglycan and collagen. When T1 values elevated and T2 values remained unchanged, it is reasonable to suggest that proteoglycan content in the matrix decreased. When both T1 and T2 values elevated, it is possible to suggest that the collagen network structure is damaged. Those findings are of significant importance in achieving an early diagnosis of OA and they may play an important role in targeted drug therapy and efficacy monitoring in vivo.
However, our research indeed has some shortcomes. First, T1 mapping scan time is too long, which brings great inconvenience to clinical work. Second, the pathogenesis and clinical manifestations of animal model and human are still different, and further study on the relevant contents of human osteoarthritis is necessary.
Acknowledgements
References
-
1.
Felson DT. Developments in the clinical understanding of osteoarthritis. Arthritis Res Ther. 2009;11(1):203. [PubMed ID: 19232065]. https://doi.org/10.1186/ar2531.
-
2.
Hunziker EB. Biologic repair of articular cartilage. Defect models in experimental animals and matrix requirements. Clin Orthop Relat Res. 1999;(367 Suppl):S135-46. [PubMed ID: 10546642].
-
3.
Kikuchi T, Sakuta T, Yamaguchi T. Intra-articular injection of collagenase induces experimental osteoarthritis in mature rabbits. Osteoarthritis Cartilage. 1998;6(3):177-86. [PubMed ID: 9682784]. https://doi.org/10.1053/joca.1998.0110.
-
4.
Paul PK, O'Byrne E, Blancuzzi V, Wilson D, Gunson D, Douglas FL, et al. Magnetic resonance imaging reflects cartilage proteoglycan degradation in the rabbit knee. Skeletal Radiol. 1991;20(1):31-6. [PubMed ID: 2000502].
-
5.
Fragonas E, Mlynarik V, Jellus V, Micali F, Piras A, Toffanin R, et al. Correlation between biochemical composition and magnetic resonance appearance of articular cartilage. Osteoarthritis Cartilage. 1998;6(1):24-32. [PubMed ID: 9616436].
-
6.
Xia Y, Moody JB, Alhadlaq H. Orientational dependence of T2 relaxation in articular cartilage: A microscopic MRI (microMRI) study. Magn Reson Med. 2002;48(3):460-9. [PubMed ID: 12210910]. https://doi.org/10.1002/mrm.10216.
-
7.
Xia Y, Moody JB, Burton-Wurster N, Lust G. Quantitative in situ correlation between microscopic MRI and polarized light microscopy studies of articular cartilage. Osteoarthritis Cartilage. 2001;9(5):393-406. [PubMed ID: 11467887]. https://doi.org/10.1053/joca.2000.0405.
-
8.
Lusse S, Claassen H, Gehrke T, Hassenpflug J, Schunke M, Heller M, et al. Evaluation of water content by spatially resolved transverse relaxation times of human articular cartilage. Magn Reson Imaging. 2000;18(4):423-30. [PubMed ID: 10788720].
-
9.
Dardzinski BJ, Laor T, Schmithorst VJ, Klosterman L, Graham TB. Mapping T2 relaxation time in the pediatric knee: feasibility with a clinical 1.5-T MR imaging system. Radiology. 2002;225(1):233-9. [PubMed ID: 12355010]. https://doi.org/10.1148/radiol.2251011461.
-
10.
Othman SF, Xu H, Royston TJ, Magin RL. Microscopic magnetic resonance elastography (microMRE). Magn Reson Med. 2005;54(3):605-15. [PubMed ID: 16088876]. https://doi.org/10.1002/mrm.20584.
-
11.
Watrin-Pinzano A, Ruaud JP, Olivier P, Grossin L, Gonord P, Blum A, et al. Effect of proteoglycan depletion on T2 mapping in rat patellar cartilage. Radiology. 2005;234(1):162-70. [PubMed ID: 15564387]. https://doi.org/10.1148/radiol.2341030394.
-
12.
Mosher TJ, Smith H, Dardzinski BJ, Schmithorst VJ, Smith MB. MR imaging and T2 mapping of femoral cartilage: in vivo determination of the magic angle effect. AJR Am J Roentgenol. 2001;177(3):665-9. [PubMed ID: 11517068]. https://doi.org/10.2214/ajr.177.3.1770665.
-
13.
Keenan KE, Besier TF, Pauly JM, Han E, Rosenberg J, Smith RL, et al. Prediction of glycosaminoglycan content in human cartilage by age, T1rho and T2 MRI. Osteoarthritis Cartilage. 2011;19(2):171-9. [PubMed ID: 21112409]. https://doi.org/10.1016/j.joca.2010.11.009.
-
14.
Nissi MJ, Rieppo J, Toyras J, Laasanen MS, Kiviranta I, Nieminen MT, et al. Estimation of mechanical properties of articular cartilage with MRI - dGEMRIC, T2 and T1 imaging in different species with variable stages of maturation. Osteoarthritis Cartilage. 2007;15(10):1141-8. [PubMed ID: 17513137]. https://doi.org/10.1016/j.joca.2007.03.018.
-
15.
Li W, Du H, Scheidegger R, Wu Y, Prasad PV. Value of precontrast T(1) for dGEMRIC of native articular cartilage. J Magn Reson Imaging. 2009;29(2):494-7. [PubMed ID: 19161210]. https://doi.org/10.1002/jmri.21658.