1. Background
Sulfur dioxide is a non-flammable, non-explosive, and colorless gas that causes a taste in concentrations of 0.3 to 1 mg/L in the air. It has a strong, irritating odor in high concentrations of 3 mg/L (1). SO2 is turned in the atmosphere into more stable end-products (2). Sulfur dioxide is emitted into the atmosphere from the combustion of sulfur-containing fossil fuels and industrial activities (3, 4).
SO2 emissions have adverse effects on human health such as respiratory problems and lung diseases (5, 6). SO2 can also have environmental consequences such as acid rain, which causes an increase in the acidity of lakes and rivers, the acidification of soils, damage to tree foliage and agricultural crops, and the corrosion of buildings and monuments (7).
Various technologies such as wet scrubbing or dry sorption methods have been developed for the removal of sulfur dioxide (4, 8). Because of their simplicity and relatively low cost, dry sorption methods are more economical than wet technologies (4). The adsorption process is a suitable method for the removal of pollutants from gaseous and aqueous environments (9-11). Dry processes, including the physical adsorption process, can be a promising strategy to remove SO2 due to its unique benefits such as low energy consumption for the adsorbent regeneration, relatively simple adsorbent design compared to the design of a chemical reactor, and few problems in waste disposal (12).
Many different adsorbents including activated carbon, alumina, graphite, rice husk ash, metal surfaces, and natural and synthetic zeolites have been applied for SO2 removal (13-19). Luo et al. reported that single gas adsorption results show that zeolite is more effective for SO2, NO, and CO2 removal among the four adsorbents (20). Due to its abundance and low cost, clinoptilolite has been used often in adsorption (21). Ivanova and Koumanova studied the modification of natural clinoptilolite from Bulgaria with salt solutions and examined the samples obtained for SO2 adsorption (22). Erdogan Alver found that the SO2 adsorption capacities of the clinoptilolite samples were superior to those of the C2H4 adsorptions (4).
Iron oxide is also common in the desulfurization process due to economic considerations and optimized dynamic properties (23). The use of nanosized iron oxide increases the efficiency of SO2 uptake due to an increased ratio of surface area to volume, it gets also properties which don’t exist in macroscopic size (24). Abbasi et al. used TiO2 nanoparticles in the removal and sensing of SO2 (25). Arcibar-Orozco et al. found that iron nanoparticles of about 3 - 4 nm enhanced the SO2 adsorption capacity by about 80% by providing well-dispersed reactive centers (26).
2. Objectives
The purpose of this study was to investigate the efficiency of sulfur dioxide removal by iron oxide nanoparticles deposited on clinoptilolite zeolite.
3. Methods
3.1. Preparation of the Adsorbent
Clinoptilolite zeolite was purchased from the Afrazand Company. It was in the form of granulated natural clinoptilolite with an average diameter of 1 - 2 mm. Ten grams of iron oxide nanoparticles (purity 99.5%) was added to one liter of distilled water containing Erlenmeyer and suspended by an ultrasonic device as a homogenous suspension. The iron oxide nanoparticle suspension was added to 200 g of granulated zeolite and placed in a shaker for 2 hours, then slowly dried in an oven at 80ºC for 10 hours. Finally, for the activation of the nanoparticles, a composite of iron oxide nanoparticles supported on clinoptilolite was heated in the oven at 300ºC for 2 hours (27).
3.2. Characterization of the Adsorbent
Scanning electron microscopy (SEM) was used to determine the surface morphology of the prepared samples (28). It was taken in a model 30 scanning electron microscope manufactured by XL Philips. The Fourier-transform infrared spectroscopy (FT-IR) was used to investigate the chemical bonds and functional groups attached to the zeolite surface (29). FT-IR was performed with the spectrum GX model apparatus, made in America. The specific surface area of the adsorbent was determined by the BET (Brunauer - Emmet - Teller) method using a BELSORP-max (Bel Japan, Inc.) instrument (30).
3.3. Adsorption Evaluation of SO2
A glass cylinder with 19.5 mm diameter and 500 mm length was used for the experiment. One hundred grams of zeolite grains with nanoparticles and without nanoparticles was placed in the glass cylinder, separately. The sulfur dioxide gas was provided in a high-pressure cylinder, which contained 2700 ppm (purity 99.98%) of SO2 balanced with N2. The gas cylinder was equipped with a pressure regulator. By passing a gas containing SO2, its removal rate was measured by determining the output concentration. The schematic diagram of the experimental setup is shown in Figure 1.
The gas flow rate was 1200 mL/min. Sampling was conducted during current to flow at 17, 20.5, 28.5, and 40 minutes and at 25, 35, 60, and 80°C. The required temperature was supplied using a 250-watt electric heating element, which was wrapped around the chamber covering the cylinder. In order to set the exact temperature, the chamber’s temperature was continuously controlled by a sensor, which was placed inside the chamber. The sensor acted quickly when the temperature was changed. If the chamber temperature was reduced from the desired temperature, the electronic thermostat quickly turned the heater on and if it was increased from the considered temperature, the thermostat turned the heater off.
After passing through the adsorbent, the gas entered in the Midget Impinger and was passed through a solution containing H2O2 (3% vol.), for determining the amount of sulfur dioxide in the effluent. Then, the formed H2SO4 was measured by titrating with a NaOH (0.01 mol/L) solution and bromcresol green and methyl red as an indicator to determine the titration endpoint. The amount of H2SO4 was applied to calculate the total sulfur amount on the adsorbent (28). The amount of sulfur dioxide in the output current was determined according to the mentioned methods in the book of Standard Methods for the Examination of Water and Wastewater (APHA) (31).
The removal efficiency was calculated based on the reactor input and output concentration difference (Equation 1).

Each experiment was performed three times. The exhaust outlet of a diesel automobile was used as a real sample.
3.4. Instrumentation
In this study, the instruments used included the HR200 analytical sensitive scale (A & D, Japan) with precision of 0.0001 g for weighing chemicals, ultrasonic device TI-H5 model (Elma, Germany) for separating nanoparticles, water distillation device (Iran), shaker (Pars Azma company) for stirring, oven (Memmert, Germany) to heat and dry adsorbents, and the Taiwanese vacuum pump model of VC-701. The chemicals that were used for the laboratory work were from Merck, Germany with high purity.
Data analysis was performed by descriptive statistics using the SPSS software (version 21).
3.5. Adsorption Kinetics
Kinetic models of pseudo-first-order and pseudo-second-order, respectively, were calculated using linear Equations 2 and 3 (32):

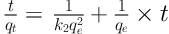
Where qe is the amount of SO2 adsorbed at equilibrium (mg/g), qt is the amount of SO2 adsorbed at time t (mg/g), and K1 and K2 are the rate constants of these two equations (33). The diagram of Log (qe-qt) against time and the diagram of t/qt versus time was plotted to examine the pseudo-first-order and pseudo-second-order, respectively, for zeolite with and without the nanoparticles at 25°C. The kinetic constants were obtained for both models by the determination of slope and intercept.
3.6. Thermodynamic Properties of Adsorption
To determine the thermodynamic parameters such as changes in standard Gibbs free energy (ΔG°), standard enthalpy (ΔH°), and standard entropy (ΔS°), Equations 4 and 5 were employed (34):


So that a straight line is achieved by plotting changes of ln K against 1/T. ΔH° and ΔS° obtained from the slope and intercept, respectively, of the linear plot.
4. Results
4.1. Characterization of Natural and Modified Zeolite
Natural and modified zeolite FTIR spectrums are shown in Figure 2A and B.
The SEM images of the natural clinoptilolite zeolite and clinoptilolite modified with iron oxide nanoparticles are illustrated in Figure 3A and B.
The BET surface area of natural clinoptilolite zeolite and modified zeolite with iron oxide nanoparticles were obtained at 19.44 m2/g and 21.7 m2/g, respectively. Adding nanoparticles of iron oxide as the active phase on the clinoptilolite zeolite has increased the specific surface area. The contact surface increases due to the small size of nanoparticles.
4.2. Effect of Temperature
The results of the effect of temperature on the removal of sulfur dioxide in natural and modified zeolite are presented in Figure 4.
The maximum values of SO2 removal efficiency is at a temperature of 25°C by natural and modified zeolite are 43.8% and 80.3%, respectively.
4.3. Effect of Contact Time
The influence of contact time on the SO2 removal efficiency was investigated and the results are provided in Figure 5.
The maximum removal efficiency of SO2 obtained in the contact time of 20.5 minutes by natural zeolite at 43.8%.
Also, the maximum removal capacity of SO2 obtained in the contact time of 28.5 minutes for modified zeolite at 80.3%.
4.4. Adsorption Kinetics
The values of K1, K2, and qe are presented in Table 1 along with the corresponding correlation coefficients (R2).
Adsorbent | Pseudo-First-Order Kinetic Model | Pseudo-Second-Order Kinetic Model | ||||
---|---|---|---|---|---|---|
K1 | qe (mg/g) | R2 | K2 | qe (mg/g) | R2 | |
Natural clinoptilolite zeolite | 0.154 | 70.1 | 0.6786 | 0.015 | 8.3 | 0.9931 |
Modified zeolite with nanoparticles | 0.182 | 365 | 0.9096 | 0.014 | 21.9 | 0.9942 |
The Kinetic Parameters for the Removal of SO2 by Natural Clinoptilolite Zeolite and Modified Zeolite with Nanoparticles at 25°C
It can be seen from Table 1 that the adsorption of SO2 on the natural zeolite and the modified zeolite with nanoparticles follows the pseudo-second-order kinetic model because the linearity percent of this equation is more than the other equation.
4.5. Adsorption Thermodynamics
The thermodynamic parameters including changes in standard Gibbs free energy (ΔG°), standard enthalpy (ΔH°), and standard entropy (ΔS°) were calculated from the variation of the thermodynamic equilibrium constant, K, at different temperatures (34). Values ΔH°, ΔS°, and ΔG° of the adsorption process are listed in Table 2.
Temperature (K) | Natural Clinoptilolite Zeolite | Modified Zeolite with Nanoparticles | ||||
---|---|---|---|---|---|---|
ΔG° (J) | ΔS° (J/mol) | ΔH° (J/mol) | ΔG° (J) | ΔS° (J/mol) | ΔH° (J/mol) | |
298 | -5476 | 2.3 | -4791 | -9622 | -20.2 | -15642 |
308 | -5499 | -9420 | ||||
333 | -5557 | -8915 | ||||
353 | -5603 | -8511 |
Thermodynamic Parameters of the Adsorption Process for SO2 at Different Temperatures
It can be seen from Table 2 that Gibbs free energy has negative values at all temperatures. The negative values of ΔG° indicate that the adsorption process of SO2 is spontaneous. The negative value of ΔS° in this table demonstrates a decreased degree of disorderliness during the adsorption of SO2 onto modified zeolite with nanoparticles. But ΔS° is positive for natural clinoptilolite zeolite, which represents an increased degree of irregularity during the adsorption process. The negative value of ΔH° confirms that the adsorption of SO2 is exothermic (35, 36).
4.6. Adsorption of Real Sample
Sulfur dioxide gas was used alone for the laboratory and synthetic sample, but to check the efficiency of the method in real conditions in the presence of other intervening factors, the diesel exhaust was used. The results showed that the efficiency of this method in actual conditions and in the presence of other intervening factors is 13.6% and 12.5% less than the laboratory sample. The removal rate obtained was 66.7% and 31.3% by modified zeolite with iron oxide nanoparticles and natural zeolite, respectively, which indicates the efficiency of this method in real terms.
5. Discussion
In the FT-IR spectra of the amended zeolite with nanoparticles, vibrations of the hydroxyl functional group bands at 3615 cm-1 can be seen. The peak at 1689 cm-1 is associated with vibrations in the Si-O band. Also, the peaks at 1515 cm-1, 1415 cm-1, and 789 cm-1 are due to Fe-O bands that appeared after the functionalization (37).
The SEM image of the natural clinoptilolite zeolite shows that the structure distribution of the zeolite is in an irregular form. In addition, the modifying zeolite with nanoparticles has increasing levels of zeolite. Images of the surface of the blended zeolite with nanoparticles also demonstrate the surface of zeolite is covered with iron oxide nanoparticles.
The results of the BET surface area indicate that the modification of natural zeolite by nanoparticles has caused an enhancement of the zeolite specific surface area, which is due to the small size of the nanoparticles, thus, increasing the contact area.
The results of the investigation of temperature indicate that the removal efficiency of sulfur dioxide is reduced with increasing temperature. It is well understood that physical adsorption decreases with increasing temperature according to Le Chatelier’s Principle. Increasing temperature enhances the movement speed of the particles and causes desorption, which can decrease the removal efficiency. Al-Harahsheh et al. studied the removal of SO2 by natural zeolite. They found that the SO2 adsorption process is exothermic on the zeolite (38). Zhou et al. studied sulfur dioxide adsorption on activated carbon. They reported that the adsorption decreases with increasing temperature and ΔH° is a negative value, which complies with the result of this study (39). Vhdat Parast et al. found that by increasing the temperature, xylene adsorption on clinoptilolite is declined (40).
The results of the investigation of the contact time demonstrate that most of the adsorption process occurs during the contact time of 20.5 and 28.5 minutes for natural zeolite and modified zeolite, respectively. By increasing the contact time, the adsorption efficiency decreases due to the restricted surface area available for adsorption. In the adsorption process, molecules physically adsorb to the adsorbent and separate from the gas stream. When all the pores of the adsorbent are filled, it is unable to adsorb more contaminant (41). Zhang et al. found that with increasing contact time, the SO2 removal efficiency by CaO as desulfurizers decreased; this is similar to the results of the current study (42). In another study by Mofidi et al. also, the removal efficiency of activated carbon for VOCs adsorption declined after a certain time (99 min) (41). Bahiraei et al. investigated the adsorption performance of γ-Fe2O3 nanoparticles for sulfide, sulfite, and thiosulfate from aqueous solutions. They reported that the adsorption process followed pseudo-second-order kinetics, which is similar with results of this study (43).
Various biological, adsorption, and catalytic procedures are used to remove pollutants from the air. These methods demonstrate the premier adsorption properties of natural zeolites such as modified clinoptilolite. Since the catalytic properties of the zeolite are not strong enough it is considered more for their adsorbing properties (44). Malakootian et al. found that the efficiency of natural and modified zeolite is relatively high in the adsorption of antibiotic tetracycline (45). On the other hand, studies have shown the catalytic properties of transition metals nanoparticles such as iron and copper in the removal of air pollutants (46). Sekhavatjou et al. investigated the removal of sulfur components from sour gas through the application of zinc and iron oxides nanoparticles (24). Also, the study by Li et al. have shown the catalytic and oxidant properties of iron nanoparticles for carbon monoxide. In their study, the conversion of carbon monoxide to carbon dioxide by 90% at 230°C was observed (27). Therefore, in the present study, the mechanisms of adsorption and catalysis can affect the efficiency of the removal of the SO2 adsorbing properties of zeolite and the strong catalytic activity of iron oxide nanoparticles. Liu et al. found that Fe additions on activated carbon showed higher SO2 adsorption capacity than the original activated carbon (28). Shen et al. investigated the removal of heavy metals by iron oxide nanoparticles. They found that the removal rate of these ions was higher using nanoparticles than a sample without nanoparticles. In addition to the iron oxide nanoparticles being very small in size, they also cover a very large surface area. Also, the functional groups on iron oxide nanoparticles increase the number of reactions. Finally, these two issues justify the high adsorption capacity of nanoparticles (47).
5.1. Conclusions
According to results, a modified zeolite with iron oxide nanoparticles has a better efficiency in adsorbing sulfur dioxide compared to unmodified zeolite due to iron oxide nanoparticles being regenerative. It can be considered as a practical, reliable, and efficient method for the removal of sulfur dioxide from the air.